7 Action Potentials
As covered in Chapter 1, the action potential is a very brief change in the electrical potential, which is the difference in charge between the inside and outside of the cell. During the action potential, the electrical potential across the membrane moves from a negative resting value to a positive value and back. Alan Hodgkin and Andrew Huxley shared the Nobel Prize in 1963[1] for their discoveries about the generation and propagation of the action potential. They shared the prize with John Eccles, who worked out how synapses work.
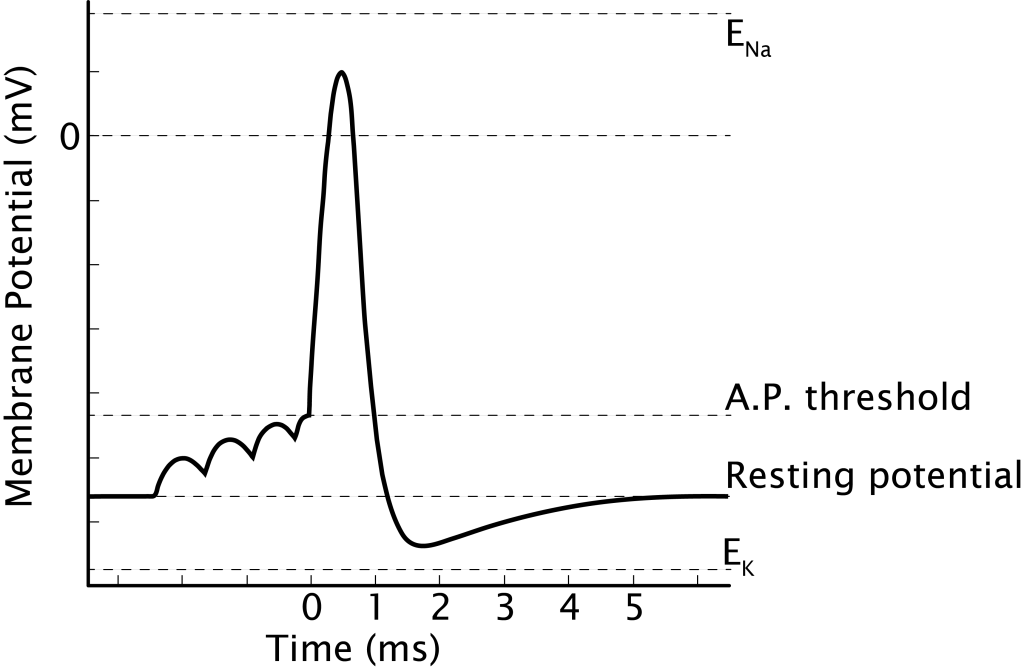
Propagation
The propagation of the action potential from the axon hillock down the axon and to the presynaptic terminal results in release of chemical neurotransmitters that communicate with a postsynaptic neuron.
Animation 7.1. The action potential moves down the axon beginning at the axon hillock. The action potential moving down a myelinated axon will jump from one Node of Ranvier to the next. This saltatory conduction leads to faster propagation speeds than when no myelin in present. When the action potential reaches the synaptic terminal, it causes the release of chemical neurotransmitter. ‘Action Potential Propagation’ by Casey Henley is licensed under a Creative Commons Attribution Non-Commercial Share-Alike (CC-BY-NC-SA) 4.0 International License. View static image of animation.
Voltage-Gated Ion Channels
The change in membrane potential during the action potential is a function of ion channels in the membrane. In the previous lessons, we have learned about the principles of ion movement and have discussed non-gated (leak) channels at rest, as well as ion channels involved in the generation of postsynaptic potentials. In this chapter, we will examine a different type of ion channel: voltage-gated ion channels. For our purposes, these channels are located primarily at the axon hillock, along the axon and at the terminal. They are necessary for the propagation of the action potential.
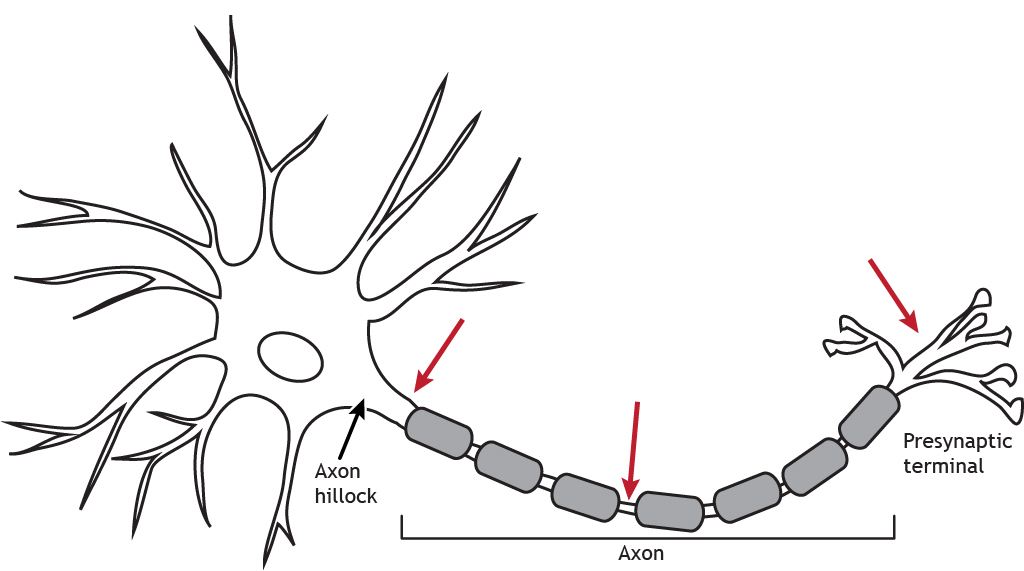
Voltage-gated channels allow ions to cross the membrane using the same ion movement principles covered in previous lessons. The main difference between voltage-gated channels and leak channels are how they are opened or “gated”. Voltage-gated channels open when the cell’s membrane potential reaches a specific value, called threshold. The neuron reaches threshold after enough EPSPs summate together.
Animation 7.2. As EPSPs summate, a result of ion movement not shown in the animation, the cell’s membrane potential will depolarize. Reaching threshold causes voltage-gated ion channels to open. Once the channels are open, ions will move toward equilibrium. In the animation, sodium ions flow inward. The dotted, blue channels represent voltage-gated sodium channels; the striped, green channels represent voltage-gated potassium channels; the solid yellow channels represent chloride channels. ‘Voltage-Gated Channel’ by Casey Henley is licensed under a Creative Commons Attribution Non-Commercial Share-Alike (CC-BY-NC-SA) 4.0 International License. View static image of animation.
The actions of the voltage-gated channels cause the different phases of the action potential
The action potential begins when the cell’s membrane potential reaches threshold, caused by the summation of EPSPs (Chapter 5). Once initiated in a healthy, unmanipulated neuron, the action potential has a consistent structure and is an all-or-nothing event. It will run through all the phases to completion[3].
The rising phase is a rapid depolarization followed by the overshoot, when the membrane potential becomes positive. The falling phase is a rapid repolarization followed by the undershoot, when the membrane potential hyperpolarizes past rest. Finally, the membrane potential will return to the resting membrane potential.
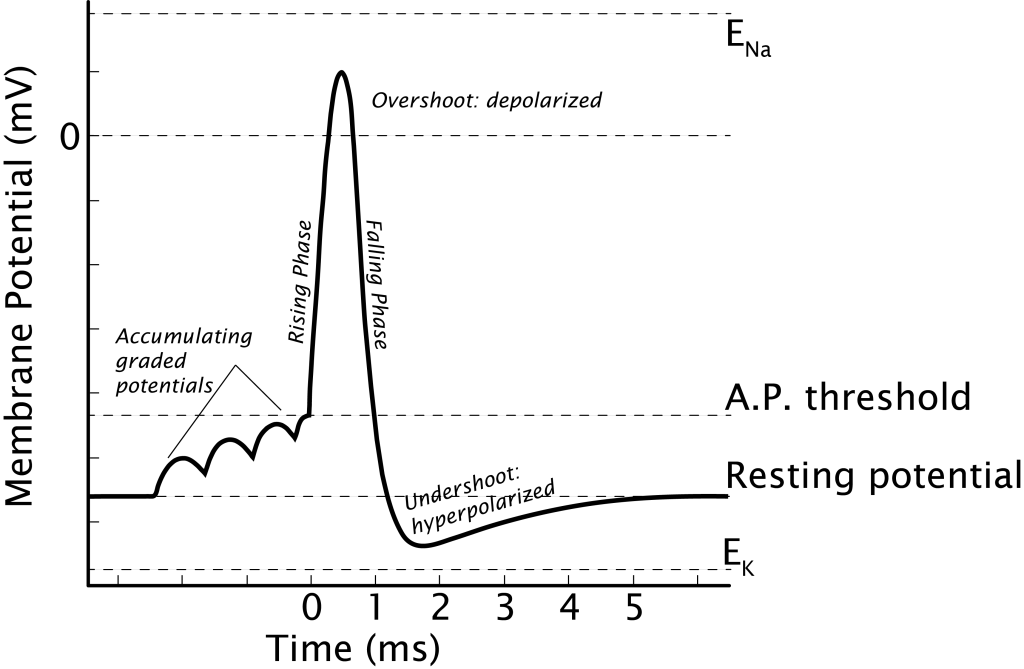
Rising Phase
The rising phase is caused by the opening of voltage-gated sodium channels. These ion channels are activated once the cell’s membrane potential reaches threshold and open immediately. The electrochemical gradients drive sodium into the cell causing the depolarization.
Animation 7.3. Voltage-gated sodium channels open once the cell’s membrane potential reaches threshold. The rapid influx of sodium results in a large depolarization called the rising phase. The dotted, blue channels represent voltage-gated sodium channels; the striped, green channels represent voltage-gated potassium channels; the solid yellow channels represent chloride channels. ‘Rising Phase’ by Casey Henley is licensed under a Creative Commons Attribution Non-Commercial Share-Alike (CC-BY-NC-SA) 4.0 International License. View static image of animation.
Falling Phase
The falling phase of the action potential is caused by the inactivation of the sodium channels and the opening of the potassium channels. After approximately 1 msec, the sodium channels inactivate. The channel becomes blocked, preventing ion flow. At the same time, the voltage-gated potassium channels open. This allows potassium to rush out of the cell because of the electrochemical gradients, taking its positive charge out of the cell, and repolarizing the membrane potential, returning the cell’s membrane potential back near rest.
Like the voltage-gated sodium channels, the voltage trigger for the potassium channel is when the cell’s membrane potential reaches threshold. The difference is that the sodium channels open immediately, whereas the potassium channels open after a delay.
Animation 7.4. After approximately 1 msec, the voltage-gated sodium channels inactivate, which prevents any further ion flow into the cell. Although the voltage-gated potassium channels are activated in response to the cell reaching threshold, their opening is delayed and occurs alone with the sodium channel inactivation. This allows an efflux of potassium ions, which causes the repolarization of the falling phase. The dotted, blue channels represent voltage-gated sodium channels; the striped, green channels represent voltage-gated potassium channels; the solid yellow channels represent chloride channels. ‘Falling Phase” by Casey Henley is licensed under a Creative Commons Attribution Non-Commercial Share-Alike (CC-BY-NC-SA) 4.0 International License. View static image of animation.
Undershoot
As the membrane potential returns to resting level, the sodium channels will de-inactivate, returning to the closed position, ready to be opened by a voltage change again. The potassium channels will also close, but they remain open long enough to cause a hyperpolarizing undershoot as potassium continues to move toward its equilibrium potential of -80 mV.
Animation 7.5. Once the cell’s membrane potential repolarizes, the voltage-gated sodium channels de-inactivate and return to their closed state. The voltage-gated potassium channels remain open long enough for the undershoot to occur as potassium continues to flow out of the cell. The dotted, blue channels represent voltage-gated sodium channels; the striped, green channels represent voltage-gated potassium channels; the solid yellow channels represent chloride channels. ‘Undershoot’ by Casey Henley is licensed under a Creative Commons Attribution Non-Commercial Share-Alike (CC-BY-NC-SA) 4.0 International License. View static image of animation.
Return to Rest
Once the voltage-gated channels close, the sodium-potassium pumps will reestablish the proper ionic concentrations needed for the electrochemical gradients. This action along with open leak channels in the membrane will return the cell to its resting membrane potential, ready to fire another action potential.
Animation 7.6. Once the voltage-gated potassium channels close, the sodium-potassium pump will work to re-establish the electrochemical gradients and return the cell to its resting membrane potential. ‘Return to Rest’ by Casey Henley is licensed under a Creative Commons Attribution Non-Commercial Share-Alike (CC-BY-NC-SA) 4.0 International License. View static image of animation.
Speed of Propagation
Presence of Myelin
The presence of myelin leads to a significant increase in action potential conduction speed compared to an unmyelinated axon. For a myelinated axon, the action potential “jumps” between Nodes of Ranvier in a process called saltatory conduction. The nodes have a high density of voltage-gated channels, and the action potential is able to skip the axon segments covered by the myelin. In an unmyelinated axon, the action potential moves in a continuous wave. In additional to the saltatory conduction process, the presence of myelin also insulates the axon, preventing charge loss across the membrane, which also increases speed of the action potential.
Animation 7.7. The action potential moves down an unmyelinated axon like a wave, opening voltage-gated channels along the length of the axon. In a myelinated axon, though, the action potential is able to skip portions of the axon that are covered by the myelin; the action potential jumps from node to node and travels further down the axon in the same amount of time. The dotted, blue channels represent voltage-gated sodium channels; the striped, green channels represent voltage-gated potassium channels. ‘Action Potential Speed’ by Casey Henley is licensed under a Creative Commons Attribution Non-Commercial Share-Alike (CC-BY-NC-SA) 4.0 International License. View static image of animation.
Diameter of Axon
The diameter of the axon also affects speed. The larger the diameter of the axon, the faster the propagation of the action potential down the axon. A larger axon leads to less resistance against the flow of ions, so the sodium ions are able to move more quickly to cause the regeneration of the action potential in the next axon segment.
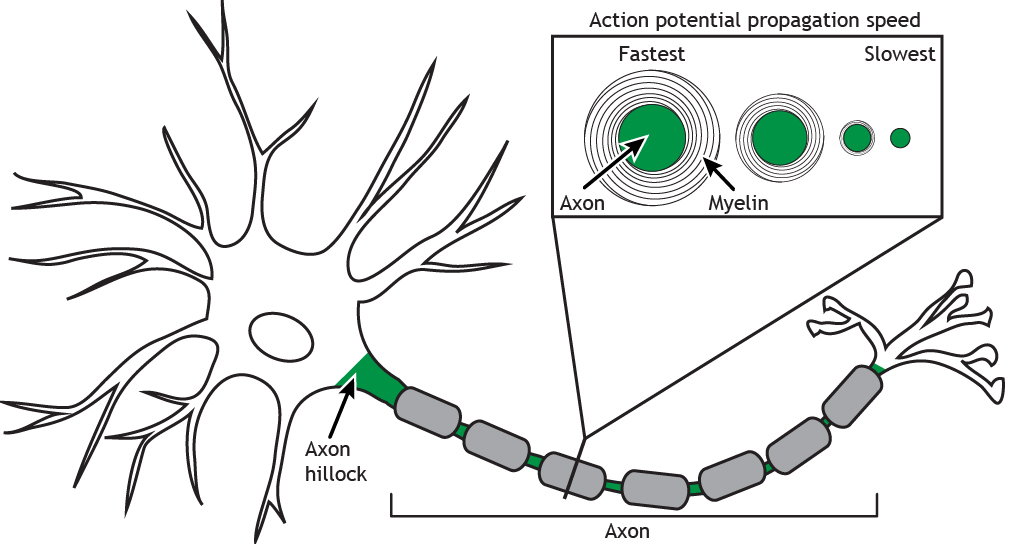
Direction of Propagation
The action potential moves down the axon due to the influx of sodium depolarizing nearby segments of axon to threshold.
Animation 7.8. A voltage change that reaches threshold will cause voltage-gated sodium channels to open in the axonal membrane. The influx of sodium causes the rising phase of the action potential, but the ion flow also depolarizes nearby axon regions. As the depolarization reaches threshold, the action potential moves down the axon. The dotted, blue channels represent voltage-gated sodium channels; the striped, green channels represent voltage-gated potassium channels. ‘Action Potential Movement’ by Casey Henley is licensed under a Creative Commons Attribution Non-Commercial Share-Alike (CC-BY-NC-SA) 4.0 International License. View static image of animation.
Action potentials only move in one direction, though, from the cell body to the presynaptic terminal. The refractory period keeps the action potential from moving backward down the axon. As the action potential moves from one Node of Ranvier to the next, the inactivated sodium channels in the previous axon segment prevent the membrane from depolarizing again. Therefore, the action potential can only move forward toward axon segments with closed sodium channels ready for rising phase depolarization.
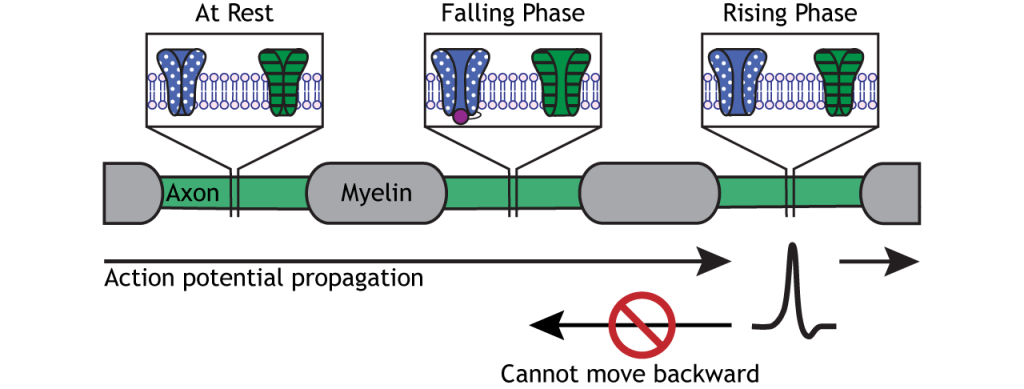
Speed of Firing Rate
Stimulus Strength
The strength of a stimulus needs to be encoded by the neurons. We need to be able to perceive the difference, for example, between a dim light and a bright one. The frequency or rate of action potential firing informs the nervous system of stimulus strength.
Since the height of the action potential is always the same for a given neuron, the strength of the stimulus is determined by the frequency of action potential firing. A weak stimulus would cause fewer action potentials to be fired than a strong stimulus.
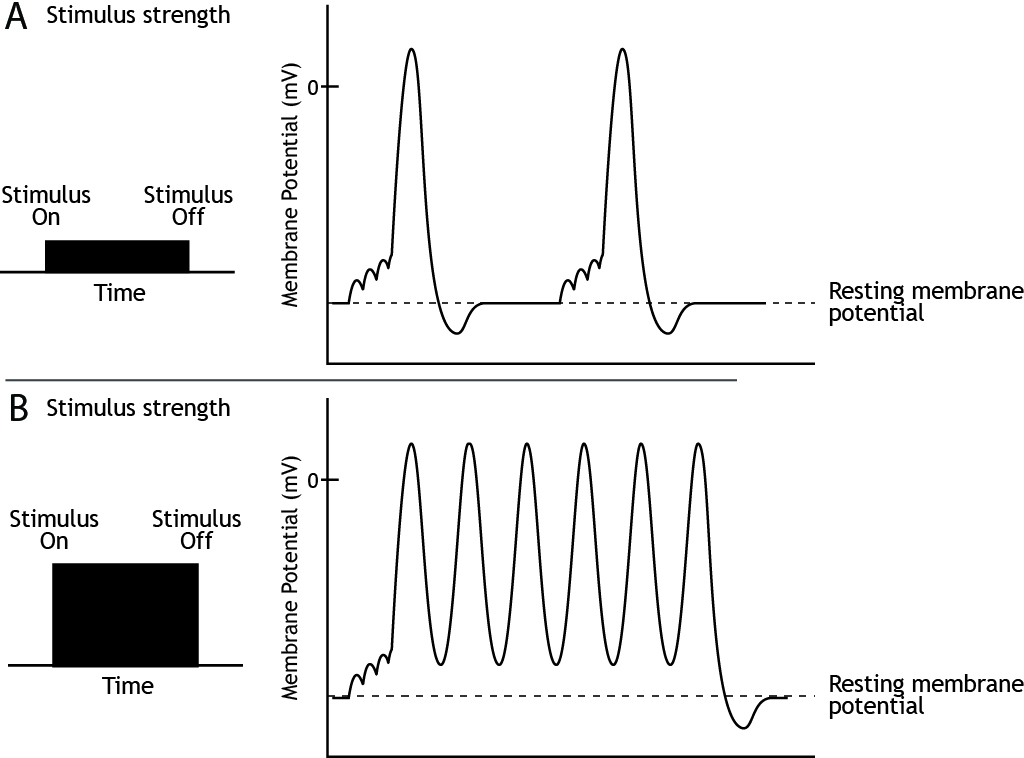
The Absolute Refractory Period
At some point, even if the stimulus continues to increase in strength, the neuron cannot fire at a higher frequency. This is the cell’s maximum firing rate. The maximum firing rate of a cell is determined by the status of the ion channels in the neuronal membrane during the different phases of the action potential. During the absolute refractory period, a second action potential cannot be fired under any circumstances regardless of the strength of the stimulus. The voltage-gated sodium channels are either open (during the rising phase) or inactivated (during the falling phase). Therefore, the cell cannot fire faster than the time it takes to pass through the absolute refractory period.
The Relative Refractory Period
When the cell repolarizes and the voltage-gated sodium channels de-inactivate and return to a closed state, the cell is again able to fire another action potential. However, during the end of the falling phase and the during the undershoot, voltage-gated potassium channels are still open. During the undershot, while the neuron is hyperpolarized, a larger-than-normal stimulus is needed to make the cell reach threshold again. This segment of the action potential is called the relative refractory period. Action potentials can be fired, but a stronger stimulus is needed than when the cell is at rest.
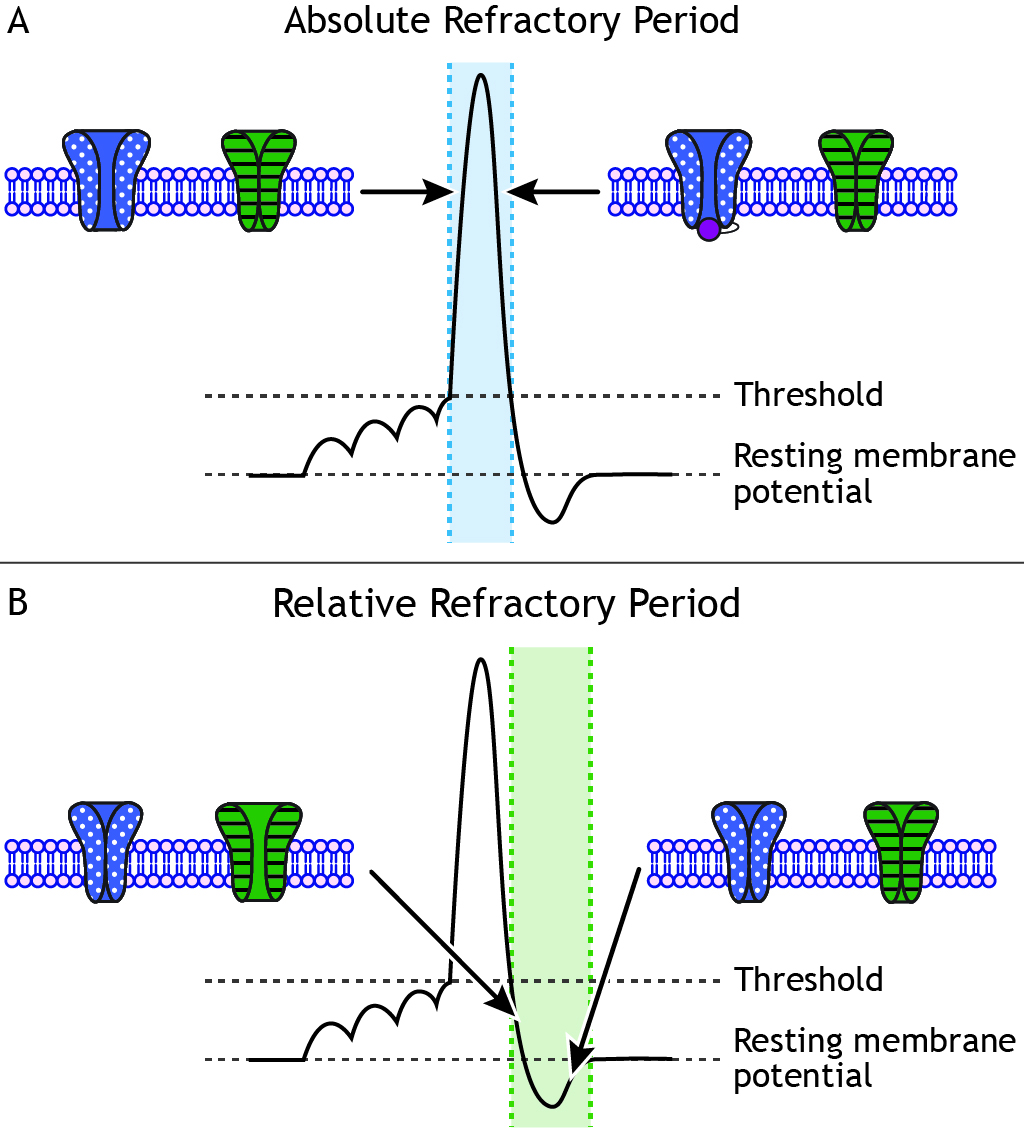
Action Potential Characteristics Can Change
For a given cell, all action potentials have the same characteristics; they depolarize to the same membrane potential value and take the same amount of time. However, different neurons may exhibit different action potential characteristics. Likewise, if a neuron has a change in its environment, like altered extracellular ion concentrations, the shape of the action potential would change due to a change in the electrochemical gradients. For example, if the external concentration of sodium is decreased, the equilibrium potential of sodium, as well as the strength of the electrochemical gradients will change, which will result in a slower rate of rise and a lower amplitude of the action potential.
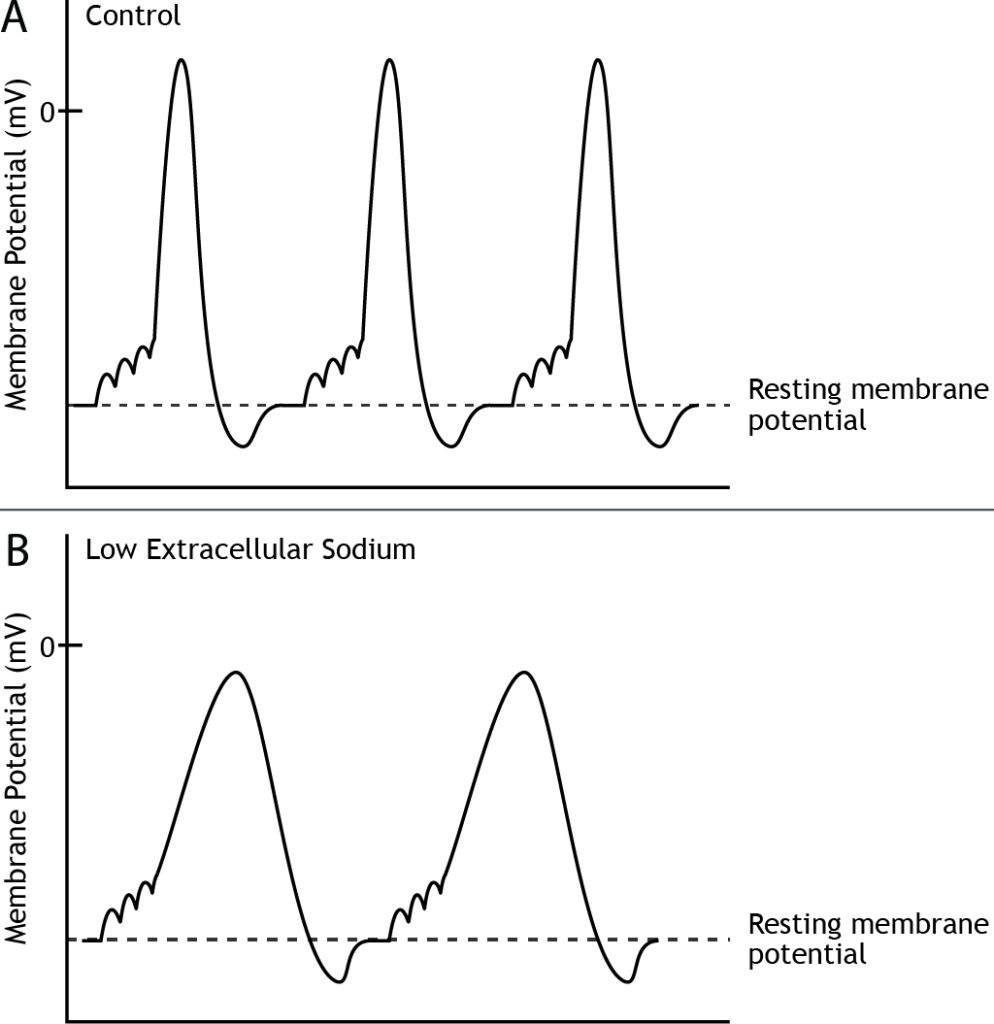
Key Takeaways
- The voltage-gated ion channels are located along the axon hillock and axon; they open in response to the membrane potential reaching a threshold value
- The rising phase of the action potential is a result of sodium influx
- The falling phase of the action potential is a result of potassium efflux
- Action potentials are all-or-none (postsynaptic potentials are graded)
- Action potential have the same height of depolarization for a given cell under typical conditions, but can change if extracellular conditions change
- Speed of propagation relies on presence and thickness of myelin and diameter of axon
- Action potential travel in one direction due to the presence of inactivated voltage-gated sodium channels
- Stimulus strength is coded by frequency of action potential firing
- The neuron cannot fire a second action potential during the absolute refractory phase
- The neuron can fire a second action potential during the relative refractory phase, but it requires a stronger stimulus than when the neuron is at rest
Test Yourself!
Try the quiz more than once to get different questions!
- From memory, draw an action potential diagram, label each phase, identify the change in membrane potential (depolarization, repolarization, and hyperpolarization), and label threshold.
- From memory and for each phase of the action potential, draw a diagram of a neuronal membrane that includes the voltage-gated ion channels in their correct state (i.e., open, closed, inactivated).
- Compare and contrast non-gated (leak) channels and voltage-gated channels.
Video Lecture
Attributions
This chapter was adapted from “Action Potentials” in Foundations of Neuroscience by Casey Henley which is licensed under a Creative Commons Attribution-NonCommercial-ShareAlike 4.0 International License.
- https://www.nobelprize.org/prizes/medicine/1963/summary/ ↵
- HODGKIN, A., HUXLEY, A. Action Potentials Recorded from Inside a Nerve Fibre. Nature 144, 710–711 (1939). https://doi.org/10.1038/144710a0 ↵
- HODGKIN AL, HUXLEY AF. A quantitative description of membrane current and its application to conduction and excitation in nerve. J Physiol. 1952 Aug;117(4):500-44. doi: 10.1113/jphysiol.1952.sp004764. PMID: 12991237; PMCID: PMC1392413. ↵