14 Neurotransmitter Action: G-Protein-Coupled Receptors
G-protein-coupled receptors (GPCRs), also called metabotropic receptors, are membrane-bound proteins that activate G-proteins after binding neurotransmitters. Like ionotropic receptors, metabotropic receptors are primarily located along the dendrites or cell body, but they can be present anywhere along the neuron if there is a synapse. Metabotropic receptors are also important for receiving incoming information from other neurons. GPCRs have slower effects than ionotropic receptors, but they can have long-lasting effects, unlike the brief action of a postsynaptic potential.
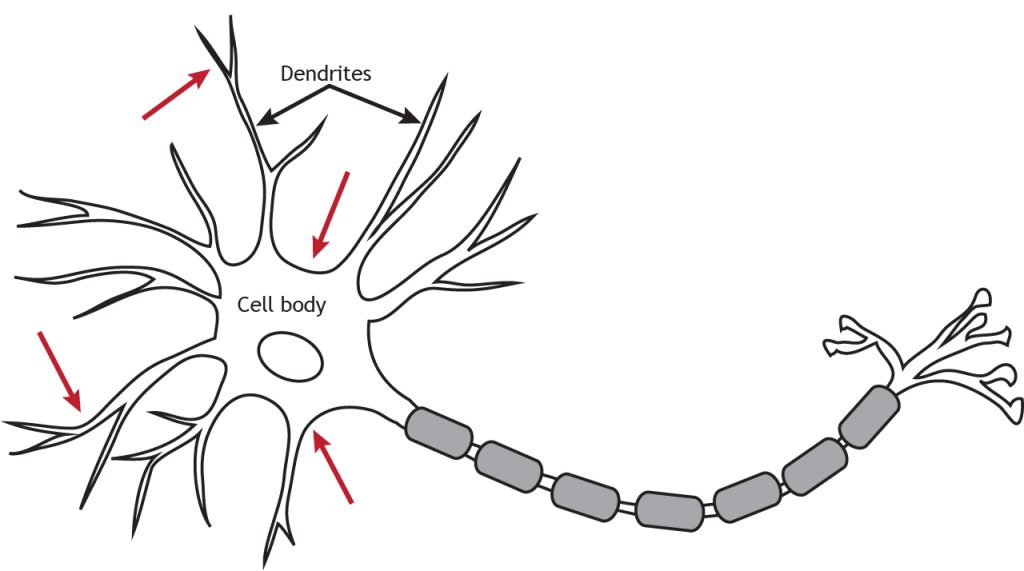
G-Proteins
G-proteins are enzymes with three subunits: alpha, beta, and gamma. In the resting state of the G-protein complex, the alpha subunit is bound to a GDP molecule. There are multiple types of alpha subunits, and each initiate different cellular cascades in the neuron.
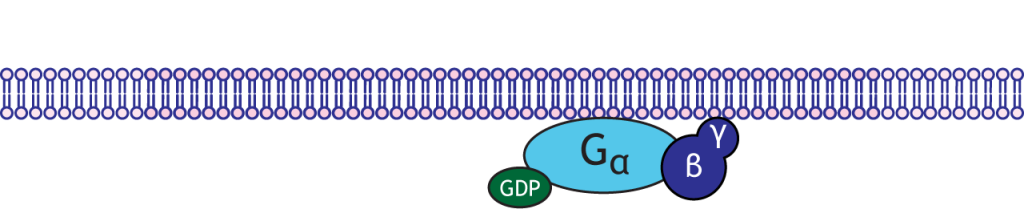
G-Protein Coupled Receptors
When a neurotransmitter binds to a GPCR, the receptor is able to interact with an inactivated G-protein complex. The complex that binds is specific to the receptor; different metabotropic receptors for the same neurotransmitter can have different effects in the cell due to which G-protein binds. Once coupled to the receptor, the GDP molecule is exchanged for a GTP molecule, and the G-protein becomes activated.
Animation 14.1. Neurotransmitter binding to a G-protein-coupled receptor causes the inactivated G-protein complex to interact with the receptor. The GDP molecule is then exchanged for a GTP molecule, which activates the G-protein complex. ‘G-protein Binding’ by Casey Henley is licensed under a Creative Commons Attribution Non-Commercial Share-Alike (CC BY-NC-SA) 4.0 International License. View static image of animation.
After activation, the G-protein complex will separate into the alpha-GTP subunit and the beta-gamma subunit. Both components can alter the function of effector proteins in the cell. Effector protein functions can range from altering ion permeability across the membrane by opening ion channels to initiating second messenger cascades. Second messenger cascades can have long-term, widespread, and diverse cellular effects including activation of cellular enzymes or altering gene transcription.
Animation 14.2. Once activated, the G-protein complex will separate into the alpha-GTP subunit and the beta-gamma subunit. These subunits can stimulate or inhibit effector proteins within the cell. ‘G-protein Effects’ by Casey Henley is licensed under a Creative Commons Attribution Non-Commercial Share-Alike (CC BY-NC-SA) 4.0 International License. View static image of animation.
Cellular Effects of G-Proteins
Open Ion Channels – Beta Gamma Subunit
Metabotropic receptors can indirectly open ion channels; this process is slower than ionotropic receptors
In certain situations, the activated beta-gamma subunit can open or close ion channels and change membrane permeability. Muscarinic acetylcholine receptors in the heart use this pathway. When acetylcholine binds to a muscarinic receptor in the heart muscle fiber, the activated beta-gamma subunit opens a type of potassium channel called G-protein-coupled inwardly-rectifying potassium (GIRK) channel, hyperpolarizing the cell. This inhibitory effect explains why acetylcholine or an agonist like atropine slow the heart rate.
Animation 14.3. Some GPCRs, like the muscarinic acetylcholine receptors in the heart, alter cellular permeability by opening ion channels. The activated beta-gamma subunit of the muscarinic receptor opens GIRK potassium channels and allows the efflux of potassium. ‘Beta-Gamma Ion Channels’ by Casey Henley is licensed under a Creative Commons Attribution Non-Commercial Share-Alike (CC BY-NC-SA) 4.0 International License. View static image of animation.
Second Messenger Cascades
Metabotropic receptors can alter protein function in the cell through second messenger cascades; these cascades may lead to changes in gene transcription
In addition to direct effects like the activated beta-gamma subunit opening ion channels, G-proteins can have many indirect actions in the cell through the use of second messenger cascades. The specific second messenger pathway that is activated or suppressed by G-protein action depends on the type of alpha subunit.
For example, norepinephrine can act on either alpha- or beta-adrenergic receptors. Beta-adrenergic GPCRs couple to a stimulatory G-protein, or Gs, which initiates the cyclic AMP (cAMP) second messenger system by activating the enzyme adenylyl cyclase. Alpha 2-adrenergic receptors, however, couple to an inhibitory G-protein, or Gi, and suppress the activity of adenylyl cyclase. Alpha 1-adrenergic receptors couple to a third type of G-protein, Gq, which activates the phospholipase C pathway. One neurotransmitter can, therefore, cause a wide range of cellular effects after binding to GPCRs, unlike the single function of ion flow through the ionotropic receptors. The pathway initiated by norepinephrine will depend on the type of receptor a specific cell expresses.
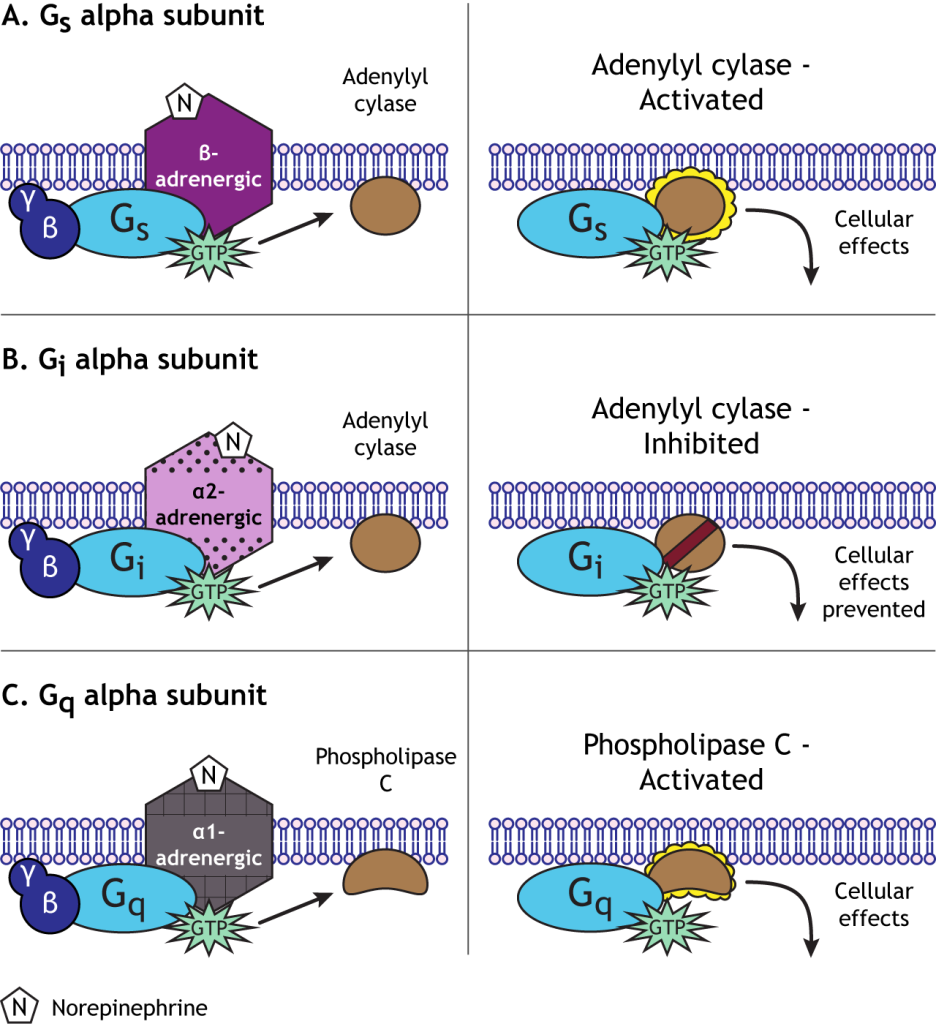
Adenylyl Cyclase / cAMP Second Messenger Cascade
The cyclic AMP (cAMP) second messenger pathway is used by many GPCRs. Activation of the pathway is caused by the Gs alpha subunit and inhibition of the pathway is caused by the Gi alpha subunit. When activated, adenylyl cyclase converts ATP to cAMP in the cytoplasm. cAMP then activates another enzyme called protein kinase A (PKA) by binding to the regulatory subunits, allowing the catalytic (functional) subunits to separate and become active. Protein kinases add a phosphate molecule to proteins, a mechanism called phosphorylation. The addition of the phosphate changes the activity of the protein and how it functions in the cell.
Animation 14.4. GPCRs that couple to the Gs alpha subunit initiate the adenylyl cyclase / cAMP pathway. The Gs subunit activates adenylyl cyclase, which then converts ATP to cAMP. cAMP binds to and activates protein kinase A (PKA), which phosphorylates proteins in the cell. ‘Adenylyl Cyclase Pathway’ by Casey Henley is licensed under a Creative Commons Attribution Non-Commercial Share-Alike (CC BY-NC-SA) 4.0 International License. View static image of animation.
The end effects of this pathway will depend on which proteins are targeted. For example, cAMP can gate ion channels and PKA can phosphorylate ion channels altering permeability and membrane potential. Phosphorylation can open the channel, or it may modulate the activity of the channel, making the channel easier to open or remain open longer.
Animation 14.5. The adenylyl cyclase / cAMP pathway can alter many cellular functions. One example is that both cAMP and PKA can open ion channels. Like ligand-gated channels, there are also cAMP-gated channels, which open after cAMP binding. PKA is able to phosphorylate and modulate ion channel function by converting ATP to ADP. ‘Second Messenger Ion Channel Action’ by Casey Henley is licensed under a Creative Commons Attribution Non-Commercial Share-Alike (CC BY-NC-SA) 4.0 International License. View static image of animation.
In addition to altering ion channel function, PKA can phosphorylate other proteins important for neuron function, such as proteins involved with neurotransmitter synthesis and release. One other critical target of PKA phosphorylation is the transcription factor CREB (cAMP response element binding-protein). Transcription factors bind to DNA in the nucleus and change the rate of gene transcription. Phosphorylation by PKA can cause CREB to initiate transcription of genes, creating new proteins for the neuron. Depending on which genes are transcribed, the effects on the neuron can be long-lasting.
Overall, neurotransmitters working through GPCRs and second messenger cascades like the adenylyl cyclase pathway can cause a diverse range of cellular effects: from opening ion channels, to changing protein activity via phosphorylation, to altering the proteins synthesized in the neuron.
Animation 14.6. PKA can phosphorylate a number of proteins involved with neuron function. It can target proteins involved with neurotransmitter synthesis, packing, and release, or it can enter the nucleus and phosphorylate CREB, a transcription factor that can initiate gene transcription and protein synthesis. ‘PKA Targets’ by Casey Henley is licensed under a Creative Commons Attribution Non-Commercial Share-Alike (CC BY-NC-SA) 4.0 International License. View static image of animation.
Phospholipase C / IP3 / DAG Second Messenger Cascade
The Gq alpha subunit initiates a separate signaling pathway in the cell by activating phospholipase C. Phospholipase C targets PIP2 (phosphatidylinositol 4,5-bisphosphate), which is a phospholipid present in the plasma membrane of the cell. PIP2 is split into two cellular molecules: IP3 (inositol 1,4,5-trisphosphate) and DAG (diacylglycerol). DAG remains in the membrane and interacts with protein kinase c (PKC). IP3 moves to the endoplasmic reticulum where it opens calcium channels and allows calcium to flow into the cytosol.
Calcium is also a second messenger in the cell. One important effect is the binding of calcium to calmodulin protein. This complex can then activate another kinase, the calcium/calmodulin-dependent protein kinase (CaMK). Both PKC and CaMK can phosphorylate specific cellular and nuclear proteins like PKA.
Animation 14.7. The Gq G-protein subunit activates phospholipase C, which converts the phospholipid PIP2 in the cell membrane into DAG, another membrane-bound molecule, and IP3, a cytoplasmic molecule. DAG can interact with PKA, initiating phosphorylation of cellular proteins. IP3 opens calcium channels in the endoplasmic reticulum, allowing calcium to flow into the cytoplasm. Calcium, another second messenger can have many cellular effects. It can bind to calmodulin, which then activates CaMK, causing phosphorylation of more protein targets. ‘IP3-DAG Pathway’ by Casey Henley is licensed under a Creative Commons Attribution Non-Commercial Share-Alike (CC BY-NC-SA) 4.0 International License. View static image of animation.
Signal Amplification
One characteristic of GPCR activation is the signal amplification that takes place. One receptor is able to activate more than one G-protein complex. The effector protein activated by the G-protein can create many second messengers, and the activated protein kinases can each phosphorylate multiple cellular proteins. This means that one neurotransmitter can have a significant effect on cellular function.
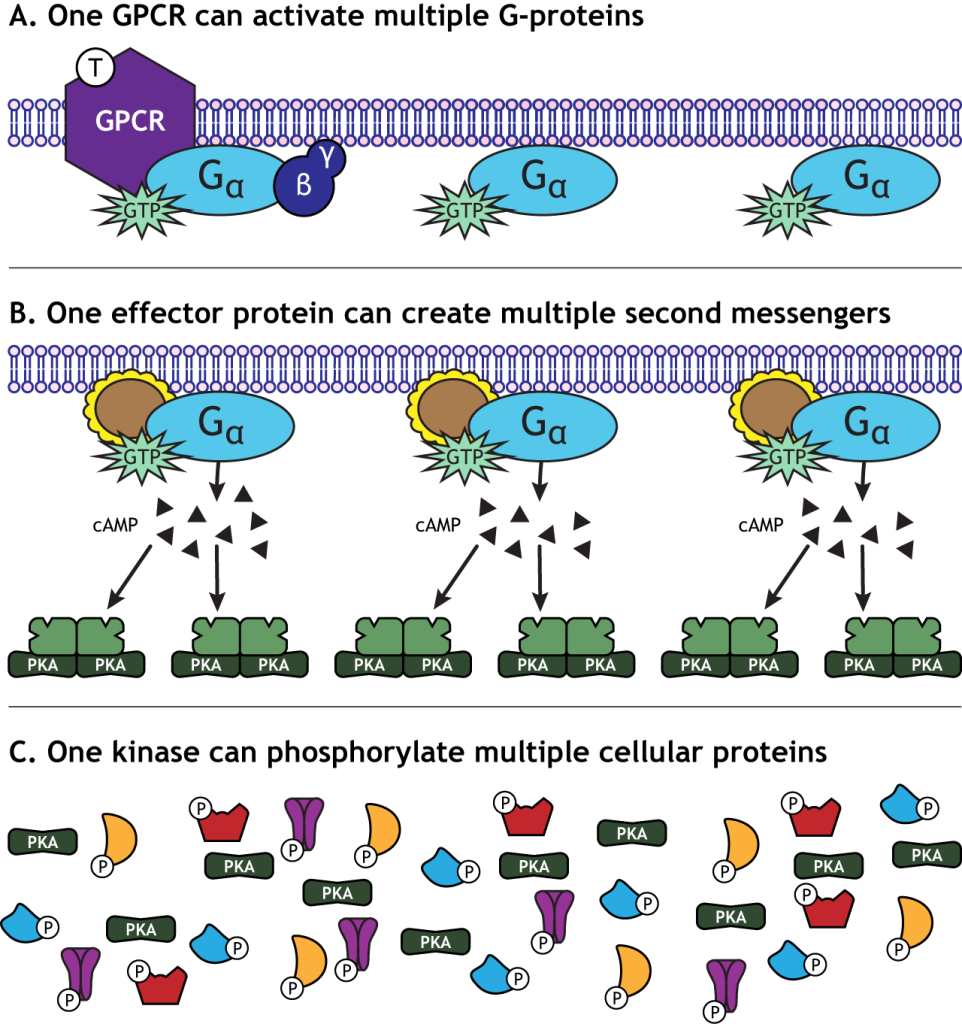
Signal Termination
Eventually, the cascade initiated by binding of the neurotransmitter to the GPCR needs to end. The alpha subunit of the G-protein is able to convert the bound GTP back to GDP after a short period of time, inactivating the G-protein. The alpha subunit will then interact with a beta-gamma subunit and stay in the resting state until activated by another GPCR. Enzymes in the cell called protein phosphatases find and remove the phosphate groups added to cellular proteins by the protein kinases. And finally, other cellular mechanisms exist to remove calcium from the cytoplasm and degrade other second messengers.
Key Takeaways
- G-protein-coupled receptors rely on the activation of G-proteins to cause cellular changes
- G-protein-coupled receptors have slower effects than ligand-gated receptors
- G-proteins can open ion channels, alter protein function via phosphorylation, and alter gene transcription
- The Gs subunit initiates the adenylyl cyclase / cAMP signaling pathway
- The Gi subunit inhibits the adenylyl cyclase / cAMP signaling pathway
- The Gq subunit initiates the phospholipase C / IP3 / DAG signaling pathway
Test Yourself!
- What are some differences between ionotropic and metabotropic neurotransmitter receptors?
Video Lecture
Attributions
This chapter was adapted from “Neurotransmitter Action: G-Protein-Coupled Receptors” in Foundations of Neuroscience by Casey Henley which is licensed under a Creative Commons Attribution-NonCommercial-ShareAlike 4.0 International License.