24 Vision: The Retina
Anatomy of the Retina
The front of the eye consists of the cornea, pupil, iris, and lens. The cornea is the transparent, external part of the eye. It covers the pupil and the iris and is the first location of light refraction. The pupil is the opening in the iris that allows light to enter the eye. The iris is the colored portion of the eye that surrounds the pupil and along with local muscles can control the size of the pupil to allow for an appropriate amount of light to enter the eye. The lens is located behind the pupil and iris. The lens refracts light to focus images on the retina. Proper focusing requires the lens to stretch or relax, a process called accommodation.
The retina is the light-sensitive region in the back of the eye where the photoreceptors, the specialized cells that respond to light, are located. The retina covers the entire back portion of the eye, so it’s shaped like a bowl. In the middle of the bowl is the fovea, the region of highest visual acuity, meaning the area that can form the sharpest images. The optic nerve projects to the brain from the back of the eye, carrying information from the retinal cells. Where the optic nerve leaves, there are no photoreceptors since the axons from the neurons are coming together. This region is called the optic disc and is the location of the blind spot in our visual field.
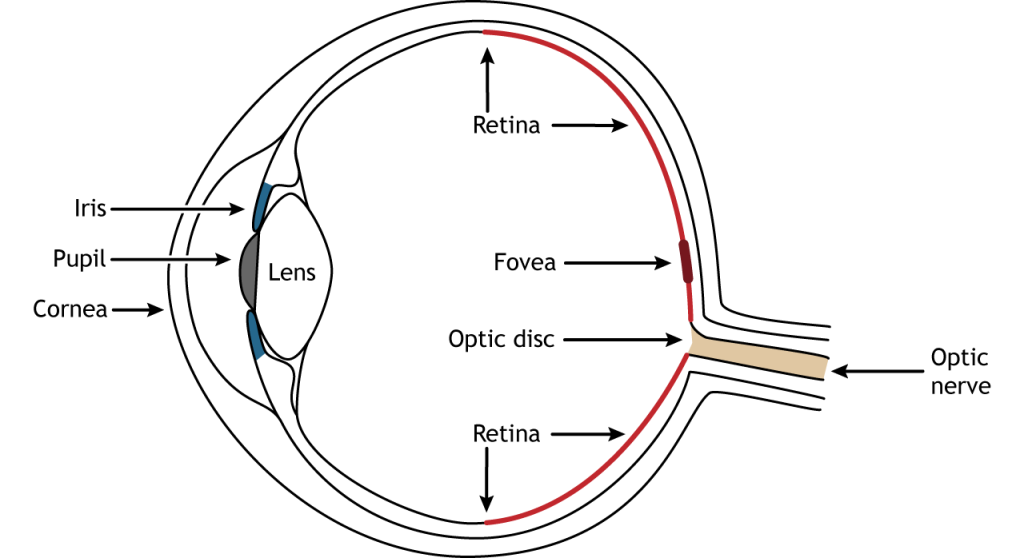
Retinal Cells
There are 5 primary types of cells in the retina
In addition to the photoreceptors, there are four other cell types in the retina. The photoreceptors synapse on bipolar cells, and the bipolar cells synapse on the ganglion cells. Horizontal and amacrine cells allow for communication laterally between the neurons.
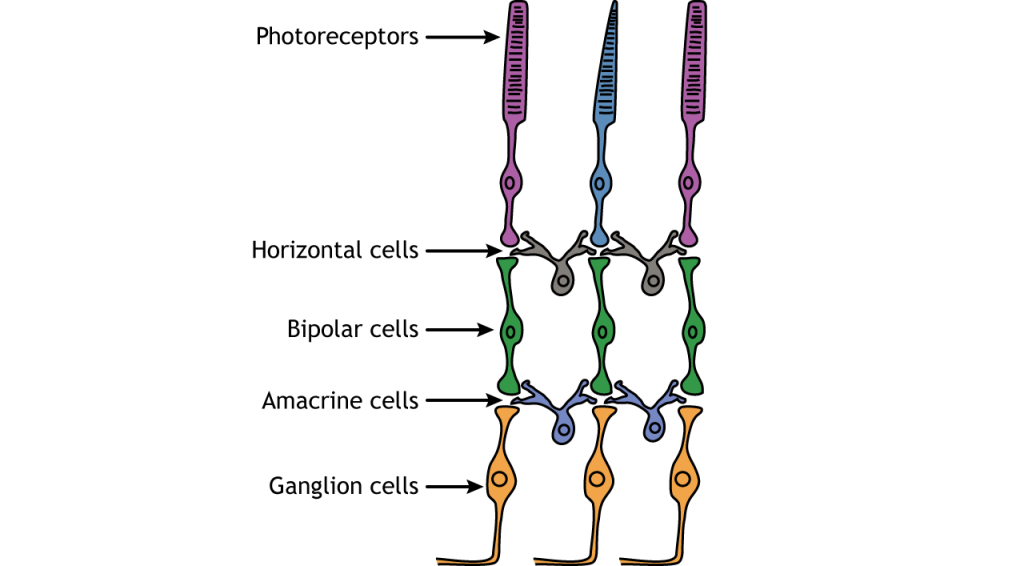
Direction of Information
When light enters the eye and strikes the retina, it must pass through all the neuronal cell layers before reaching and activating the photoreceptors. The photoreceptors then initiate the synaptic communication back toward the ganglion cells.
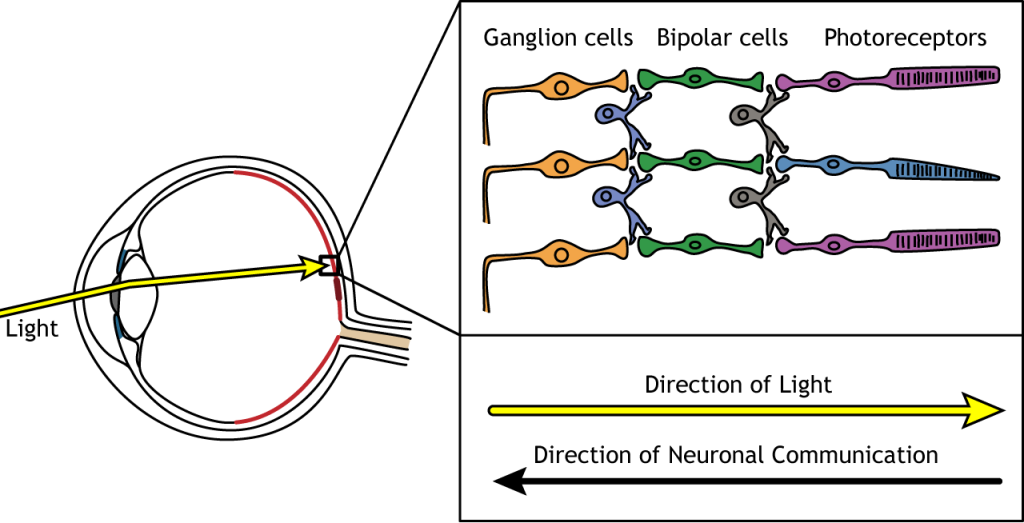
Receptors
The photoreceptors are the specialized receptors that respond to light. There are two types of photoreceptors: rods and cones. Rods are more sensitive to light, making them primarily responsible for vision in low-lighting conditions like at night. Cones are less sensitive to light and are most active in daylight conditions. The cones are also responsible for color vision.
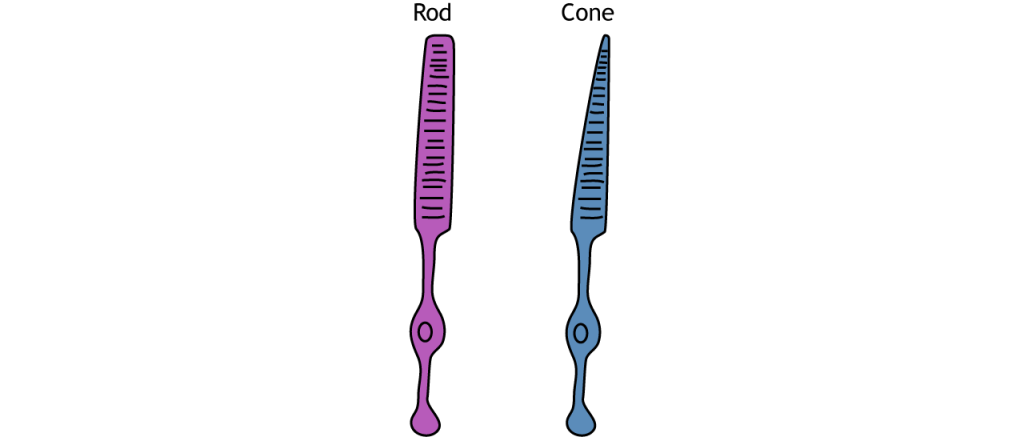
Receptor Density
In addition to having different visual functions, the rods and cones are also distributed across the retina in different densities. The cones are primarily found in the fovea, the region of the retina with the highest visual acuity. The remainder of the retina is predominantly rods. The region of the optic disc has no photoreceptors because the axons of the ganglion cells are leaving the retina and forming the optic nerve.
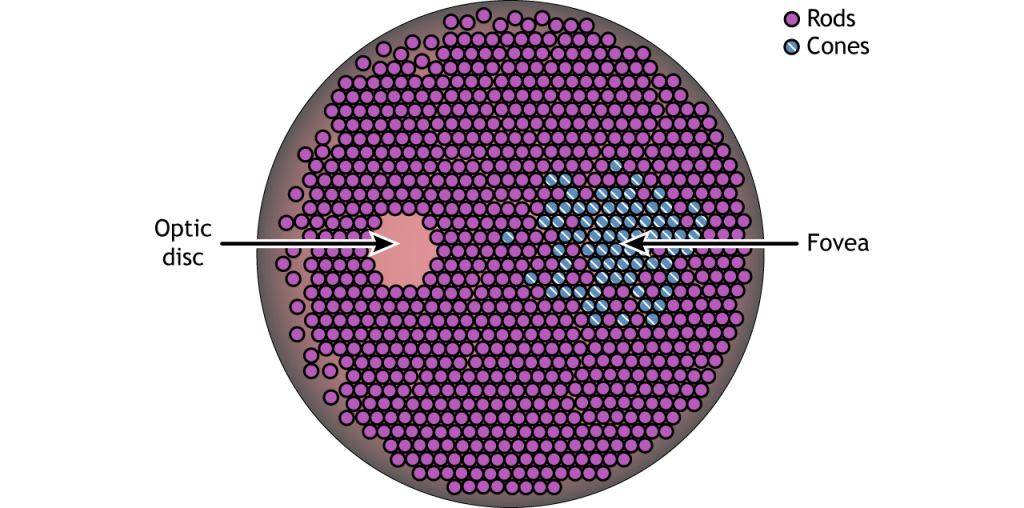
Phototransduction
Photoreceptors hyperpolarize in response to light, do not fire action potentials, and release glutamate
The photoreceptors are responsible for sensory transduction in the visual system, converting light into electrical signals in the neurons. For our purposes, to examine the function of the photoreceptors, we will A) focus on black and white light (not color vision) and B) assume the cells are moving from either an area of dark to an area of light or vice versa.
Photoreceptors do not fire action potentials; they respond to light changes with graded receptor potentials (depolarization or hyperpolarization). Despite this, the photoreceptors still release glutamate onto the bipolar cells. The amount of glutamate released changes along with the membrane potential, so a hyperpolarization will lead to less glutamate being released. Photoreceptors hyperpolarize in light and depolarize in dark. In the graphs used in this lesson, the starting membrane potential will depend on the initial lighting condition.
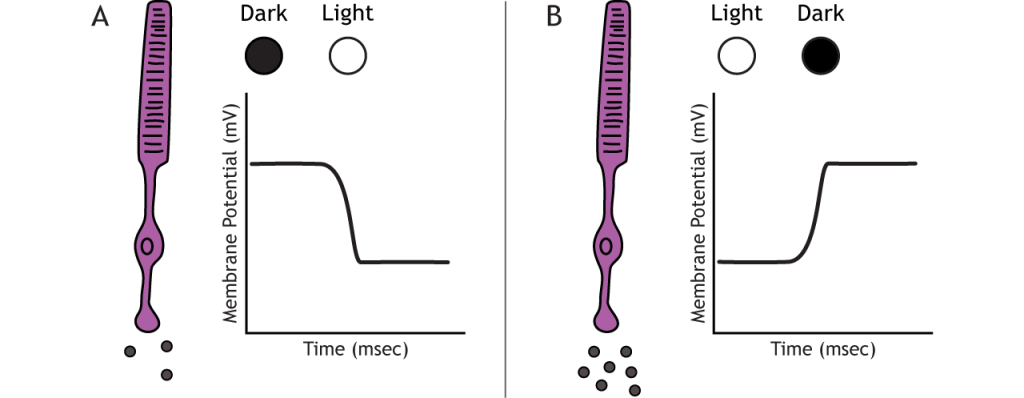
In the dark, the photoreceptor has a membrane potential that is more depolarized than the “typical” neuron we examined in previous chapters; the photoreceptor membrane potential is approximately -40 mV. Photoreceptors have open cation channels that allow the influx of sodium and calcium in the dark. These channels are gated by the presence of cyclic GMP (cGMP), a molecule important in second-messenger cascades that is present in the photoreceptor in the dark.
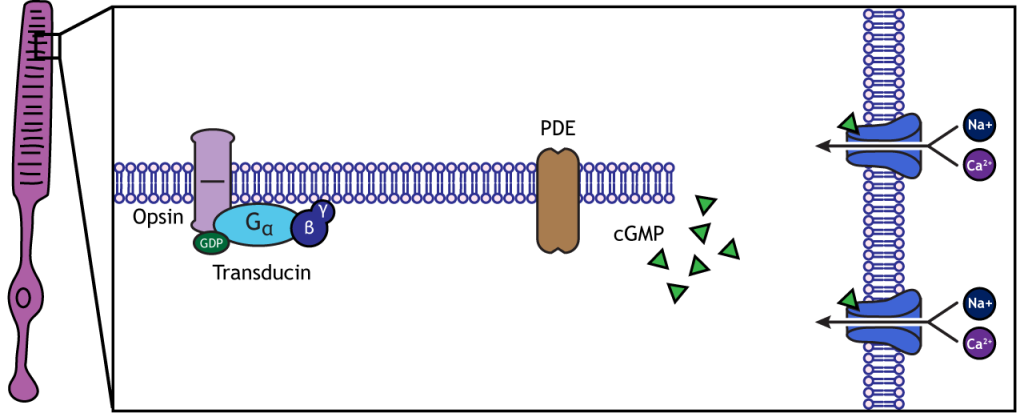
When the photoreceptor moves into the light, the cell hyperpolarizes. Light enters the eye, reaches the photoreceptors, and causes a conformational change in a special protein called an opsin. This change activates a G-protein called transducin, which then activates a protein called phosphodiesterase (PDE). PDE breaks down cGMP to GMP, and the cGMP-gated ion channels that were open in the dark close. The decrease in cation flow into the cell causes the photoreceptor to hyperpolarize.
Animation 24.1. Light reaching the photoreceptor causes a conformational change in the opsin protein, which activates the G-protein transducing. Transducin activates phosphodiesterase (PDE), which converts cGMP to GMP. Without cGMP, the cation channels close, stopping the influx of positive ions. This results in a hyperpolarization of the cell. ‘Phototransduction’ by Casey Henley is licensed under a Creative Commons Attribution Non-Commercial Share-Alike (CC BY-NC-SA) 4.0 International License. View static image of animation.
Transmission of Information within Retina
Photoreceptors synapse onto bipolar cells in the retina. There are two types of bipolar cells: OFF and ON. These cells respond in opposite ways to the glutamate released by the photoreceptors because they express different glutamate receptors. Like photoreceptors, the bipolar cells do not fire action potential and only respond with graded postsynaptic potentials.
OFF bipolar cells depolarize in the dark; ON bipolar cells depolarize in the light
OFF Bipolar Cells
In OFF bipolar cells, the glutamate released by the photoreceptor is excitatory. OFF bipolar cells express ionotropic glutamate receptors. In the dark, glutamate released by the photoreceptor activates the ionotropic receptors, and sodium can flow into the cell, depolarizing the membrane potential. In the light, the absence of glutamate causes the ionotropic receptors to close, preventing sodium influx, hyperpolarizing the membrane potential.
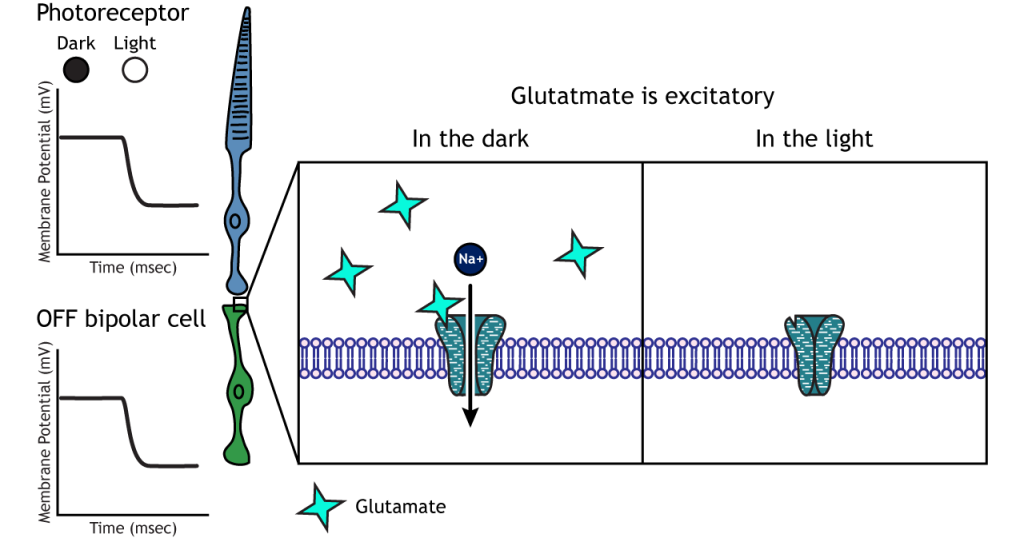
ON Bipolar Cells
In ON bipolar cells, the glutamate released by the photoreceptor is inhibitory. ON bipolar cells express metabotropic glutamate receptors. In the dark, glutamate released by the photoreceptor activates the metabotropic receptors, and the G-proteins close cation channels in the membrane, stopping the influx of sodium and calcium, hyperpolarizing the membrane potential. In the light, the absence of glutamate results in the ion channels being open and allowing cation influx, depolarizing the membrane potential.
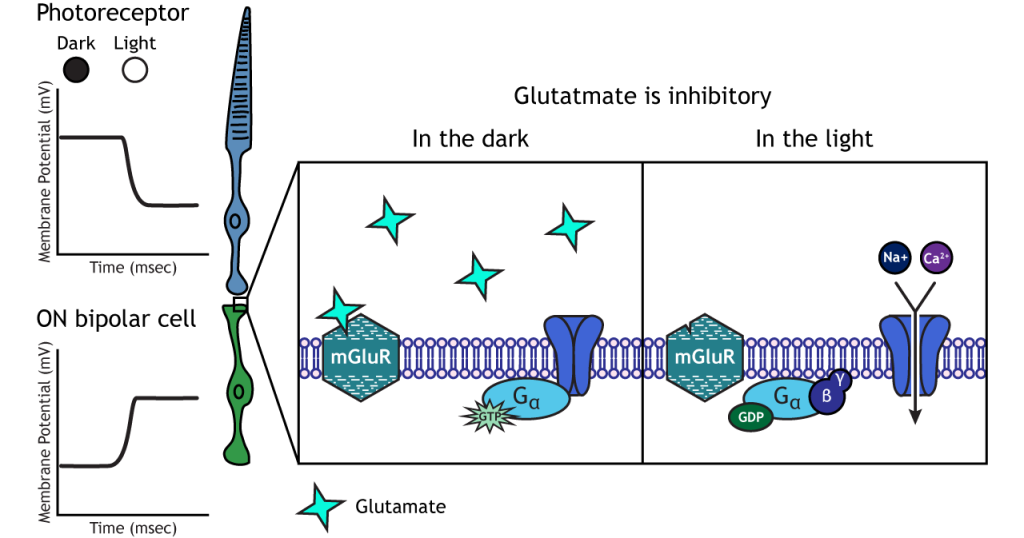
Ganglion Cells
OFF-center ganglion cells increase firing rate in the dark; ON-center ganglion cells increase firing rate in the light
OFF and ON bipolar cells synapse on OFF-center and ON-center ganglion cells, respectively. Ganglion cells are the only cell type to send information out of the retina, and they are also the only cell that fires action potentials. The ganglion cells fire in all lighting conditions, but it is the relative firing rate that encodes information about light. A move from dark to light will cause OFF-center ganglion cells to decrease their firing rate and ON-center ganglion cells to increase their firing rate.
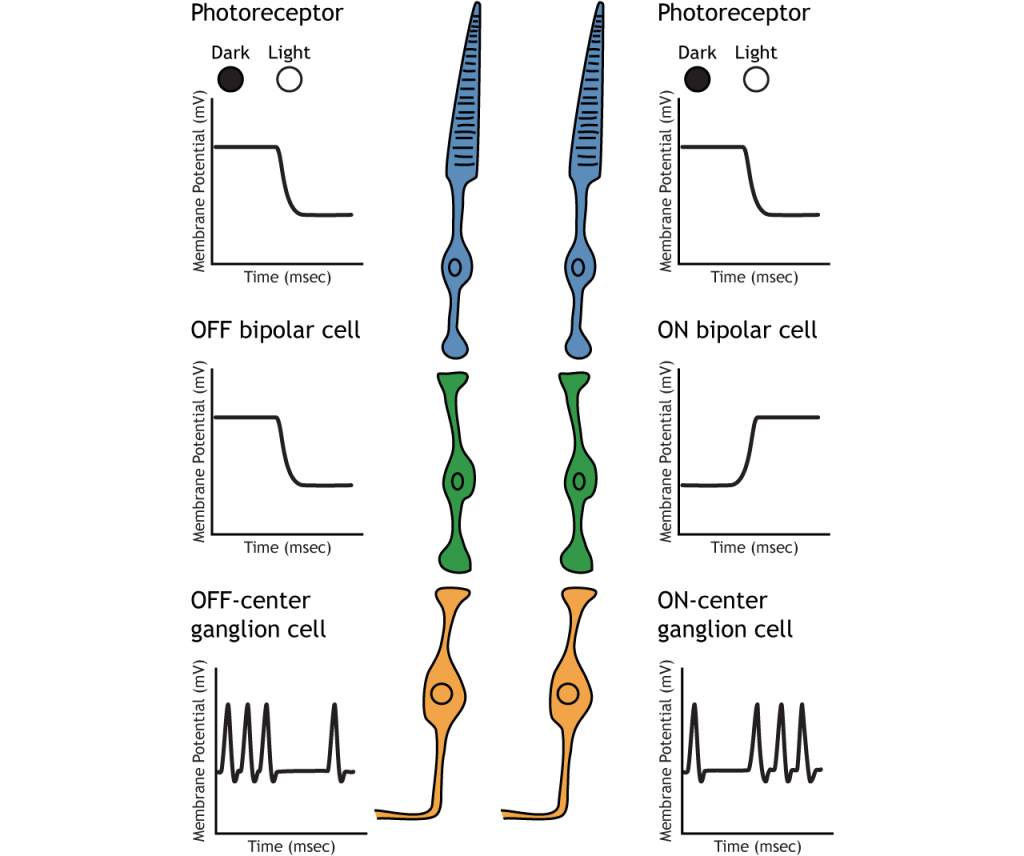
Receptive Fields
Each bipolar and ganglion cell responds to light stimulus in a specific area of the retina. This region of retina is the cell’s receptive field. Receptive fields in the retina are circular.
Size of the receptive field can vary. The fovea has smaller receptive fields than the peripheral retina. The size depends on the number of photoreceptors that synapse on a given bipolar cell and the number of bipolar cells that synapse on a given ganglion cell, also called the amount of convergence.
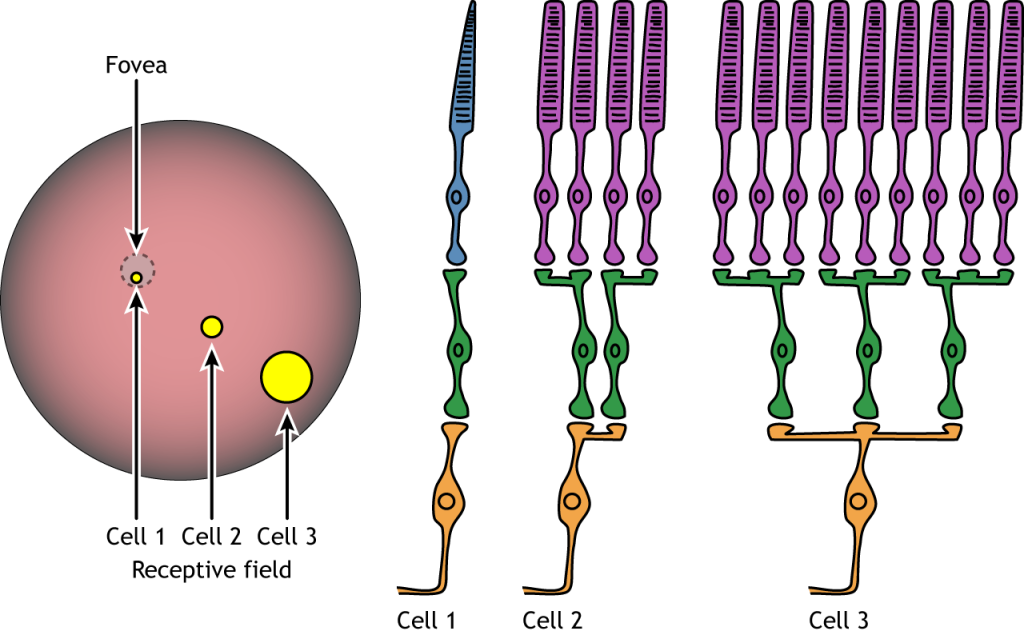
Receptive Field Example
Let’s use an example of an ON bipolar cell to look at the structure of receptive fields in the retina. The bipolar and ganglion cell receptive fields are divided into two regions: the center and the surround. The center of the receptive field is a result of direct innervation between the photoreceptors, bipolar cells, and ganglion cells. If a light spot covers the center of the receptive field, the ON bipolar cell would depolarize, as discussed above; the light hits the photoreceptor, it hyperpolarizes, decreasing glutamate release. Less glutamate leads to less inhibition of the ON bipolar cell, and it depolarizes.
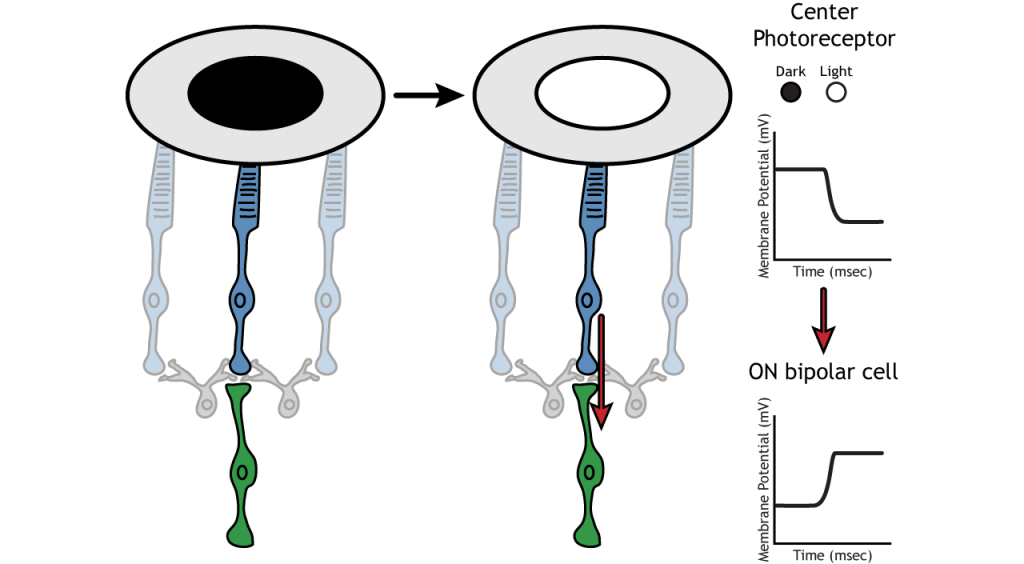
The surround portion of the receptive field is a result of indirect communication among the retinal neurons via horizontal and amacrine cells. The surround also has an opposing effect on the bipolar or ganglion cell compared to the effect of the center region. If a light spot covers the surround portion, the ON bipolar cell would respond by hyperpolarizing. The light would cause the photoreceptor in the surround to hyperpolarize. This would cause the horizontal cell to also hyperpolarize. Horizontal cells have inhibitory synaptic effects, so a hyperpolarization in the horizontal cell would lead to a depolarization in the center photoreceptor. The center photoreceptor would then cause a hyperpolarization in the ON bipolar cell. These effects mimic those seen when the center is in dark. So even though the center photoreceptor is not directly experiencing a change in lighting conditions, the neurons respond as if they were moving toward dark.
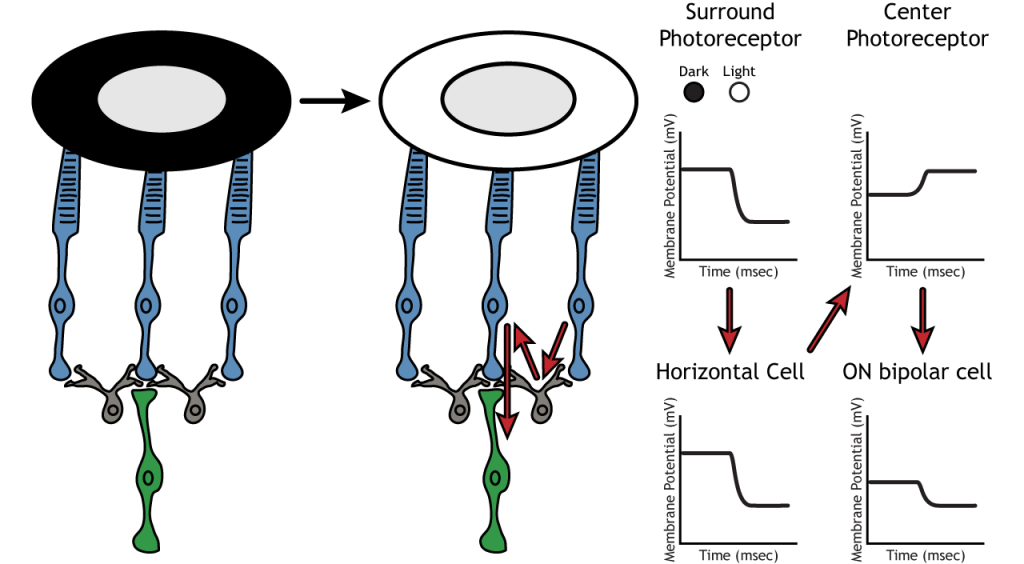
Lateral Inhibition
Lateral inhibition is critical for the enhance perception of edges and borders
The center-surround structure of the receptive field is critical for lateral inhibition to occur. Lateral inhibition is the ability of the sensory systems to enhance the perception of edges of stimuli. It is important to note that the photoreceptors that are in the surround of one bipolar cell would also be in the center of a different bipolar cell. This leads to a direct synaptic effect on one bipolar cell while also having an indirect effect on another bipolar cell.
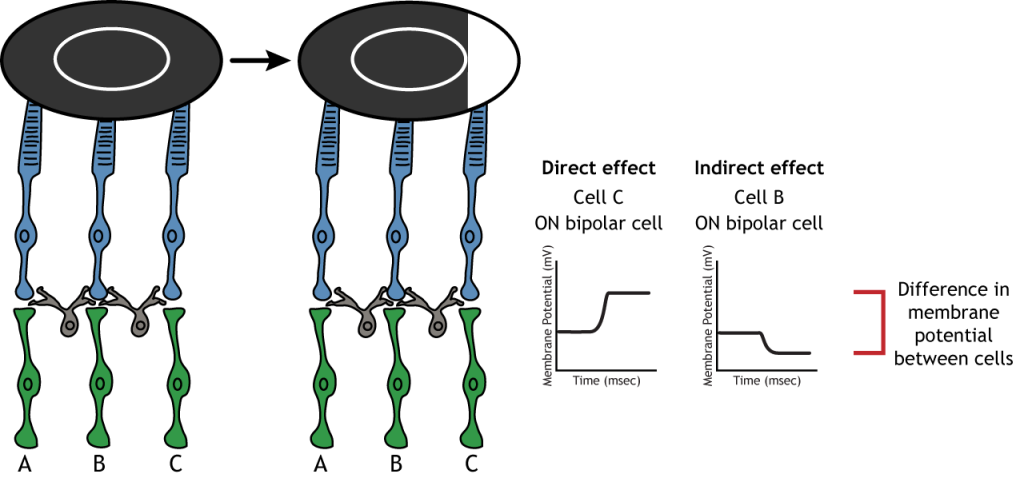
Although some of the images used here will simplify the receptive field to one cell in the center and a couple in the surround, it is important to remember that photoreceptors cover the entire surface of the retina, and the receptive field is two-dimensional. Depending on the level of convergence on the bipolar and ganglion cells, receptive fields can contain many photoreceptors.
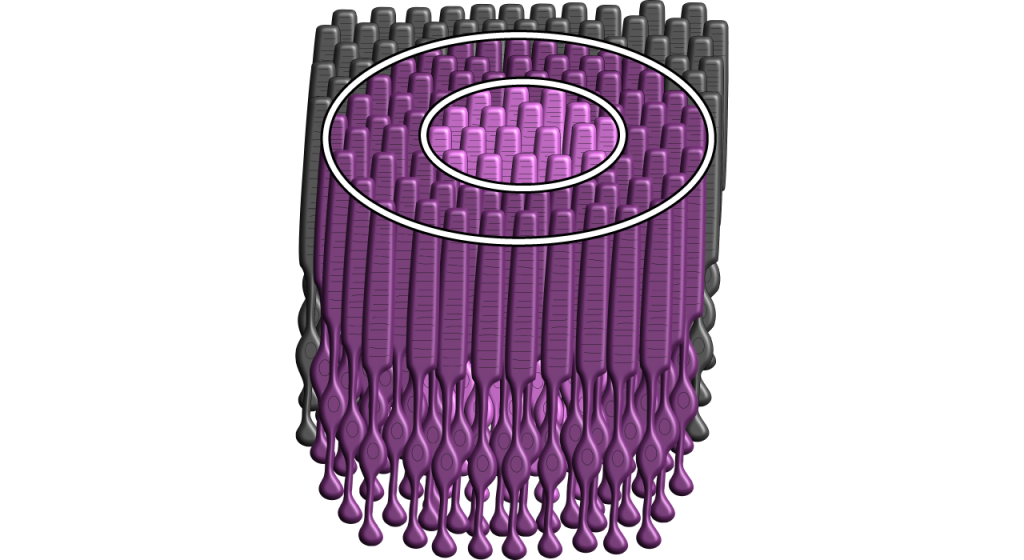
Key Takeaways
- Photoreceptors and bipolar cells do not fire action potentials
- Photoreceptors hyperpolarize in the light
- ON bipolar cells express inhibitory metabotropic glutamate receptors
- OFF bipolar cells express excitatory ionotropic glutamate receptors
- Receptive fields are circular, have a center and a surround, and vary in size
- Receptive field structure allows for lateral inhibition to occur
Test Yourself!
Video Lecture
Attributions
This chapter was adapted from “Vision: The Retina” in Foundations of Neuroscience by Casey Henley which is licensed under a Creative Commons Attribution-NonCommercial-ShareAlike 4.0 International License.